Quantum Waves and Their Uses
Notes toward a workshop on using quantum physics to measure things
I’ve agreed to do a pre-conference workshop later this summer with the general theme of using quantum mechanics to do measurements. If you read general-audience science books and articles, seeing the words “quantum” and “measurement” in the same sentence may lead you to expect a lot of philosophical wibbling about observer effects and the (non)existence of objective reality, but this is not that. On the contrary, there’s a large area of research built around using quantum-mechanical effects to make exceptionally precise and sensitive measurements of various phenomena of practical interest.
Of course, there’s a fairly trivial sense in which all of modern metrology is rooted in quantum effects, just because it makes heavy use of computers and lasers. Both of these are fundamentally quantum in nature: lasers use the stimulated emission of light by atoms to create bright and monochromatic beams, while computers rely on exploiting the band structure of semiconductors to create transistor circuits that can function as bits in a digital computer. I’ve got big long discussions of both of these in Breakfast With Einstein.
There’s a sense, though, in which those technologies are not really quantum in nature. The billions of transistors on a computer chip act as classical bits: either 0 or 1 at all times, just like a macroscopic toggle switch. Most applications of lasers treat them as essentially bright classical sources: an electromagnetic wave with a single frequency and well-defined phase.
You can do amazing things with these basically classical systems— the interference of light waves lets you measure positions with nanometer precision, and classical computers can do enormously complicated calculations at incredible speed. This enables all manner of signal-processing tricks that can pull tiny signals out of vast quantities of data.
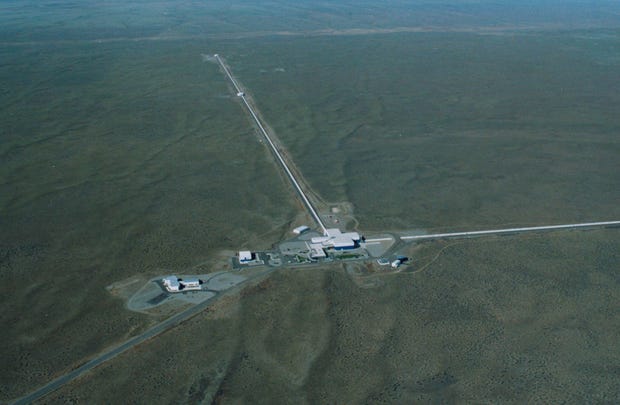
The paradigm system for this sort of quantum-based-but-classical-in-character measurement is the Laser Interferometer Gravitational-wave Observatory (LIGO). This is a giant Michelson interferometer that splits a laser beam onto two perpendicular paths several kilometers long and then recombines the beams and looks at the resulting interference pattern. The intensity of the light at the output of the interferometer is incredibly sensitive to changes in the position of the mirrors that send the light back, at a level that can detect the tiny stretching and compression of spacetime as a gravitational wave goes by.
Of course, there are a vast number of mundane effects that move the mirrors by larger distances than gravitational waves do— everything from earthquakes to passing trucks to thermal fluctuations in the coatings on the mirrors themselves— which is where the computers come in. The LIGO detectors produce a vast stream of data measuring the intensity of the output light as a function of time, and this is then fed into computer algorithms that slice and dice it in ways that let the scientists find patterns that have the right properties to be a gravitational wave and not just someone down the hall doing jumping jacks.
This system is incredibly successful, enough that despite the mind-boggling difficulty of the underlying task they have detected eighty-odd collisions of gigantic astronomical objects1, some of which have been correlated with more conventional astronomical detections (visible light, x-rays, gamma rays). It’s an astonishing achievement in terms of science and technology.
In a sense, though, this is only the crudest kind of measurement you can do with quantum-based systems; if you want to throw tech-inflected buzzwords around, you could call these “quantum 1.0” systems. They make use of quantum mechanics to generate exceptionally good classical sources for use in measurements. But there’s an opportunity to move to a “quantum 2.0” approach, where you directly exploit the quantum nature of light and matter to improve your measurements.
So, for example, you can look at the fact that material objects have wave nature, meaning that an atom is itself an oscillating system with a particular phase that depends on its energy and momentum. This wave nature allows you to produce superposition states, where the atom is in both the state “0” and the state “1” at the same time, with the two states evolving at slightly different rates. Which lets you do interference experiments like the one done by LIGO— separating a wave onto two “paths” (quote marks because the difference is just a matter of internal state) and bringing it back together— within a single quantum system2.
The result of that interference experiment lets you measure incredibly tiny perturbations to the energies of the underlying quantum states. If you apply a magnetic field, say, you can increase or decrease the energy difference between “0” and “1” by a tiny amount, which can make a big change in the output signal. This allows the construction of quantum sensors that can be used to measure magnetism in biomedical systems, or high-resolution imaging of atom-scale magnetism.
You can also physically separate the atoms onto two paths, exactly the way LIGO does with light beams, to make an atom interferometer. This has the nice property that the atoms, by virtue of having mass, are subject to gravitational forces, which like the magnetic fields in the internal superposition, affect the evolution of the two paths in different ways. This lets you make extremely sensitive measurements of gravity on a variety of length scales and at extreme sensitivity. Especially if you make these interferometers insanely large.
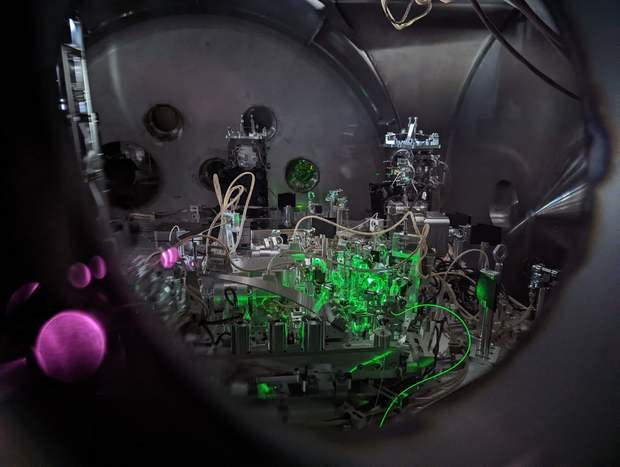
You can also look to the quantum nature of light for ways to improve measurements. A beam of light is not just a wave, it’s also a collection of photons, which have particle character. That combination introduces some uncertainty into the collective properties of the light— that pesky Heisenberg Uncertainty Principle— but also give you a bit of a loophole. If you’re willing to throw away information about one aspect of the light— the overall number of photons, say— you can trade that for an improved measurement of a complementary property— the phase of the light. This process is called “squeezing,” from the effect it has on the standard graphical representation of these states, and can be used to make beams of light that can exceed the ordinary limits on measurement.
Which, fittingly, comes back to LIGO, which is one of the world-leading experiments in this category. They’ve spent years working to introduce squeezed light into their systems to increase the measurement sensitivity, with squeezed light coming into play in the most recent data runs.
That’s a quick overview of the general idea of quantum for measurement. There are a lot of other technologies and applications that can fall under this rubric, and it’s a relatively young field. I hope it’s at least sufficient to demonstrate the general idea of this as a sensible field, and the viability of the split between quantum-based-but-classical (“quantum 1.0”) and fully quantum (“2.0”) measurement techniques3.
This is largely me thinking out loud about the classification scheme I was asked to talk about, but since I have to do that anyway, I might as well get a blog post out of it. If you want to see more of me talking to myself as I put this together, here’s a button to get it in your inbox:
And if you have questions or points of disagreement, the comments will be open:
That’s “to date” according to the FAQ page at the LIGO site, which helpfully is undated.
Note that while this uses the now-obligatory “0” and “1” labeling, this is not talking about quantum computing, though you can call these systems “qubits” if you want to impress a granting agency. The relevant measurements are all single-qubit effects, though, not anything you would normally talk about as a quantum computation.
The post title here is a nod to Albert Michelson’s “Light Waves and Their Uses,” which I’ve banged on about before.
Did you get the email I sent you after I visited the Atom Trap Trace Analyses facility in Adelaide? They use laser cooling to trap trace cosmogenic isotopes like 39Ar or 83Kr and count them.
"wibbling" ← thanks for the new2me word
Also, lol @ fn1